Cytochrome-c aptamer functionalized Pt nanoclusters for enhanced chemodynamic therapy
Abstract
Catalysis-based chemodynamic therapy (CDT) is an emerging cancer treatment strategy which uses a Fenton-like reaction to kill tumor cells by catalyzing endogenous hydrogen peroxide (H2O2)2) into a toxic hydroxyl radical (⋅⋅OH). The performance of CDT is greatly dependent on PDT agent. Herein, mitochondria-targeting Pt nanoclusters were synthesized using cytochrome c aptamer (CytcApt) as template. The obtained CytcApt-PtNCs can produce ⋅⋅OH by H2O2 under the acidic conditions. Moreover, CytcApt-PtNCs could kill 4T1 tumor cells in a pH-dependent manner, but had no side effect on normal 293T cells. Therefore, CytcApt-PtNCs possess excellent therapeutic effect and good biosafety, indicating their great potential for CDT.
1. Introduction
Traditional tumor chemotherapy employs chemodrugs, which has strong side effects for normal cells. In recent years, introducing biocompatible and nontoxic nanoparticles into tumor tissues to catalyze/trigger specific in situ chemical reactions have received great interest.1,2 These unique intratumoral chemical reactions are defined as “catalytic chemical-reaction nanomedicine”, which refers to chemical reactions catalyzed by nanoparticles for efficient cancer therapy.3 This emerging research areas shows a wide range of applications including chemodynamic therapy (CDT), gas therapy, tumor hypoxia alleviation, and so on.4,5,6,7 CDT catalyzes chemical reactions in the special tumor microenvironment (TME) including low pH, excessive hydrogen peroxide (H2O2)2), and low catalase expression.8 Specifically, functional nanomaterials act as catalysts to trigger Fenton or Fenton-like reaction under the mildly acidic conditions of TME, catalyzing H2O2 to produce highly toxic hydroxyl radicals (⋅⋅OH), which can induce tumor cells death.9 As the nature of TME is slightly acidic and has excessive H2O2, therefore, the reaction is only induced in tumor tissues, but suppressed in normal tissue microenvironment, which could safely, efficiently, and specifically treat cancer.10
Numerous metal nanoparticles have been designed to trigger intratumoral Fenton or Fenton-like reaction for CDT, such as Fe2+2+/Fe3+3+, Cu++/Cu2+2+, Mn2+2+/Mn4+4+, and so on.11,12,13 Unlike nanoparticles, nanoclusters are composed of several to dozens of atoms, and the size is less than 2 nm.14 Due to the ultra-small size, metal nanoclusters have excellent biocompatibility, ease of conjugation, and renal clearance, which has been widely applied in cancer nanotheranostics.15,16,17 Platinum nanoclusters (PtNCs) have shown excellent Fenton catalytic activity in colorimetric detection of metal ions and colorimetric biosensor.18,19,20 To the best of our knowledge, the application of PtNCs in the CDT has not yet been investigated.
In order to improve the efficiency of CDT in tumor cells, a specific targeting molecule need to be linked to the nanoclusters. Aptamer is a single-stranded DNA or RNA oligonucleotides, which can act like antibodies and bind multiple biological targets (proteins, DNA, and small molecules) with high affinity and specificity.21 The small size of aptamer is favoring the anchoring of ultra-small metal nanoclusters, and has no effect on the stability of nanoclusters.22,23,24,25 For example, metal nanoclusters modified by a cytochrome c aptamer (CytcApt) showed specific binding to cytochrome c, which is located in the inner mitochondrial membrane.26,27,28 Nanoclusters modified by CytcApt can specifically accumulate in the mitochondria of tumor cells because the concentration of H2O2 is highest in the mitochondria of tumor cells.29 Therefore, preparing mitochondria-targeted CDT agent could significantly improve the tumoricidal efficiency.
Herein, for the first time, mitochondria-targeted PtNCs was synthesized using CytcApt as template, which could be used as a CDT agent to achieve greater therapeutic effect on tumor cells. 5,5′-tetramethylbenzidine (TMB) indicator and electron spin resonance (ESR) spectroscopy were used to examine the generation of ⋅OH by H2O2 under the acidic conditions to improve the Fenton catalytic activity of CytcApt-PtNCs. The highly toxic ⋅OH can induce cell death, and CytcApt can target PtNCs to mitochondria with highest H2O2 concentration. Therefore, excessive ⋅OH in mitochondria triggered by CytcApt-PtNCs could lead to tumor cells death. To assess the production of ⋅OH in tumor cells, we measured the production of ⋅OH of 4T1 cells after treated by CytcApt-PtNCs. Cell viability evaluation proved the biocompatibility of CytcApt-PtNCs for normal 293T cells. Further, the performances of CDT of CytcApt-PtNCs for tumor cells were assessed in 4T1 cells. The staining method of live-dead cells also proved the excellent anti-tumor effect of CytcApt-PtNCs as CDT nanoagent.
2. Materials and Methods
2.1. Materials
Disodium hydrogen phosphate, sodium dihydrogen phosphate, and sodium chloride were acquired from Sinopharm Chemical Reagent (Shanghai, China). Chloroplatinic acid hexahydrate (H2PtCl6⋅6H2O, 99%), dimethylamine borane (DMAB), 2′,7′-dichlorodihydrofluorescein diacetate (DCFH-DA), Calcein AM, Propidium Iodide (PI), and 3-(4,5-dimethyl-2-thiazolyl)-2,5-diphenyl-2-H-tetrazolium bromide (MTT) were purchased from Sigma-Aldrich. Fetal bovine serum (FBS), Dulbecco’s Modified Eagle’s medium (DMEM), RPMI 1640 cell culture medium, and Trypsin-EDTA (0.25%) were purchased from Beyotime Biotechnology (Shanghai, China). CytcApt sequence: 5′-HS-(CH2)6-GCACTGGTCGGCCATGGGTAGCGACGGTCCCTAACGTTT-3′ was synthesized by Genscript Biotechnology Co. Ltd. (Nanjing, China). All chemical reagents are of analytical grade and were used as received without further purification.
2.2. Synthesis of cytcApt-PtNCs
100μmol of CytcApt was mixed with 1mmol of H2PtCl6⋅6H2O in a solution of phosphate buffer (pH=4.4) and equilibrated for 12h at room temperature until the reaction solution changed to yellow color. The mixture was then reduced by adding 10mmol DMAB followed by equilibration for 12h. The obtained CytcApt-PtNCs were stored at 4∘C in the dark and used for the following experiment. In addition, H2PtCl6 and CytcApt with different concentrations were studied to optimize the properties of the PtNCs.
2.3. Apparatus and characterization
Electron spin-resonance spectroscopy (ESR) was performed on a BRUKER ER073 spectrometer. Transmission electron microscope (TEM) images of CytcApt-PtNCs were obtained using a TALOS F200X (FEI, USA) instrument. UV-vis spectra were obtained using a UV-2450 spectrophotometer (Shimadzu, Kyoto, Japan). Circular dichroism (CD) spectra were collected on a JASCO instrument using a 1mm path length cuvette, three scans were collected for each sample. Confocal images were acquired using a TCS SP8 microscope (Leica, Germany).
2.4. Catalytic activity evaluation
The generation of ⋅OH of CytcApt-PtNCs was evaluated using 3,3′,5,5′-tetramethylbenzidine (TMB) assay, which is oxidized by ⋅OH to form into oxidized TMB (oxTMB) with a maximum absorbance at 652nm.30 After 30min, the catalytic activity of CytcApt-PtNCs to TMB was tested using a UV-Vis spectrophotometer.
In addition, we further used 5, 5-dimethyl-1-pyrroline N-oxide (DMPO) as trapping agent to measure the production of ⋅OH of CytcApt-PtNCs by ESR spectrometry.31
2.5. Cell culture
The 4T1 cells (mouse breast cancer cells) were seeded in RPMI 1640 medium supplemented with 10% FBS, penicillin (100μg/mL), and streptomycin (100μg/mL) in 5% CO2 at 37∘C. The 293T (human embryonic kidney cells) were cultured similarly with the exception that RPMI 1640 medium was replaced with DMEM.
2.6. Cell viability evaluation
The 4T1 or 293T cells were seeded in a 96-well plate (1×103 cells per well) and cultured overnight at 37∘C under 5% CO2. After 24h cultivation, different concentrations of CytcApt-PtNCs were added to the wells. After 24h cultivation, MTT solution was added to the wells for another 4h incubation. Finally, the MTT absorption was detected using enzyme mark instrument at 490nm.
2.7. Intracellular reactive oxygen species detection
Reactive Oxygen Species (ROS) can be measured by DCFH-DA probe, which can be oxidized by ROS and then emits green fluorescence. After co-culturing with 32 μM CytcApt-PtNCs for 4h, the cells were washed with PBS and cultivated with DCFH-DA for 30min. Finally, the ROS level of cells was obtained by confocal fluorescence microscopy.
2.8. Live/Dead cells co-staining assay
4T1 cells (1×104 cells/mL) were incubated for 24h, then cultivated with 32μM CytcApt-PtNCs for 4h. Then cells were co-stained with Calcein AM and PI, and washed again with PBS three times. Finally, the live/dead cells were recorded by confocal fluorescence microscopy.
3. Results and Discussion
3.1. Preparation and characterization of CytcApt-PtNCs
The different amount of reducing agents DMAB has a great influence on the valence state and particle size of the synthesized CytcApt-PtNCs, which are closely related to their catalytic ability. The catalytic performance of CytcApt-PtNCs was optimized by studying different molar ratios of Pt, DNA, and DMAB. CytcApt-PtNCs was synthesized under different Pt:DMAB ratios and tested for their ability to catalyze the generation of ⋅OH from H2O2 by the TMB method. By comparing the characteristic absorption peaks at 650nm, Fig. 1 showed that the catalytic ability was the best at a ratio of 1:7.
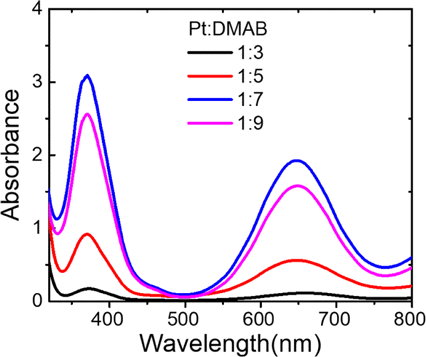
Fig. 1. The catalytic ability of different ratios of Pt:DMAB during CytcApt-PtNCs synthesis.
The X-ray photoelectron spectroscopy (XPS) analyses of CytcApt-PtNCs were shown in Fig. 2(a), the peaks at approximately 74.5 and 71.3eV corresponds to metallic Pt, and the peaks at approximately 76 and 72.6eV corresponds to Pt (II). The generation of Pt (II) can be attributed to the formation of PtCl2 precipitation.32,33 The size and morphology of CytcApt-PtNCs were analyzed by high-resolution TEM. As shown in Fig. 2(b), the CytcApt-PtNCs displayed a well-defined spherical morphology with a well distribution. Statistical calculation presented that the average size of CytcApt-PtNCs was 1.83nm±0.41nm (Fig. 2(c)), further verified that ultrasmall nanoclusters have been successfully synthesized.
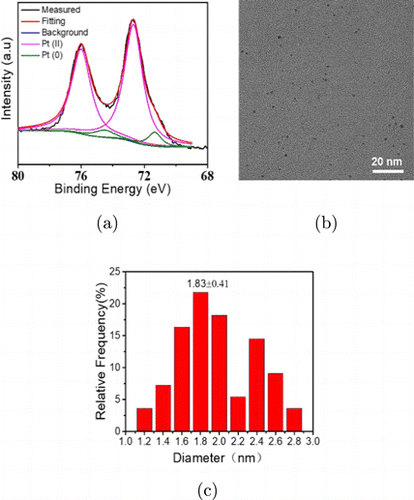
Fig. 2. The XPS spectra (a), high-resolution TEM image (b) and distribution of particle size (c) of CytcApt-PtNCs.
The structure of the aptamer before and after synthesis of CytcApt-PtNCs was measured by CD spectra. The peak intensity showed a slight decrease and there was a redshift in the peak position from 284 nm to 289 nm upon conjugation of the PtNCs to the CytcApt (Fig. 3). This implied that the secondary structure of the CytcApt switched from a “parallel” type to a “folded” type, which may play a role in fine tuning their cell target specificity.34
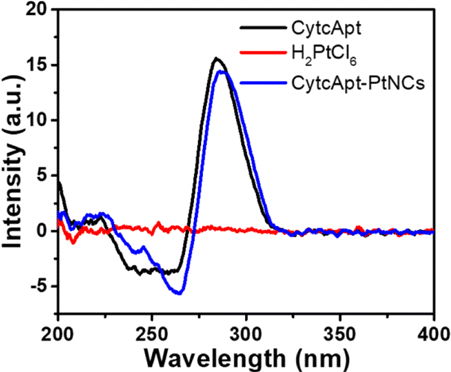
Fig. 3. Circular dichroism (CD) spectra of CytcApt and CytcApt-PtNCs.
3.2. Catalytic activity evaluation of CytcApt-PtNCs
In this process, H2O2 was catalyzed by CytcApt-PtNCs to generate ⋅OH for TMB oxidation, which was observed by the characteristic absorption peaks at 652nm with time (Fig. 4). Meanwhile, this ability of CytcApt-PtNCs to produce ⋅OH was showed in a pH-dependent manner, with best activity at pH5.4. No change was found at normal environment (pH7.4), which confirms the safety of CytcApt-PtNCs in the slight alkaline conditions of normal tissues. However, acidic environment (pH6.5 and pH5.4) accelerated the speed of ⋅OH generation, which indicated that CytcApt-PtNCs could produce a great number of ⋅OH in the acidic TEM.
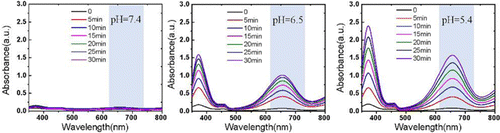
Fig. 4. UV-vis absorbance spectra of ox-TMB in the presence of CytcApt-PtNCs.
In addition, the generation of ⋅OH catalyzed by CytcApt-PtNCs was assessed by ESR with DMPO as free-radical spin-trapping agent. As shown in Fig. 5, a typical peak pattern of DMPO/⋅OH was recorded, and the signal intensity was increased when the pH value was lowered from 7.4 to 5.4. These results further confirmed that the CytcApt-PtNCs can efficiently generate ⋅OH and presented the low-pH dependent tendency, which may be attributed to the generation of more ⋅OH in a lower acidic environment. TMB assay and ESR spectroscopy experiments confirmed that CytcApt-PtNCs, acidic conditions, and H2O2 are the three necessary factors for producing ⋅OH. The generation of increasing amount of ⋅OH at lower pH values may be beneficial to the acceleration of the Fenton-like reaction in a more acidic environment of tumor.
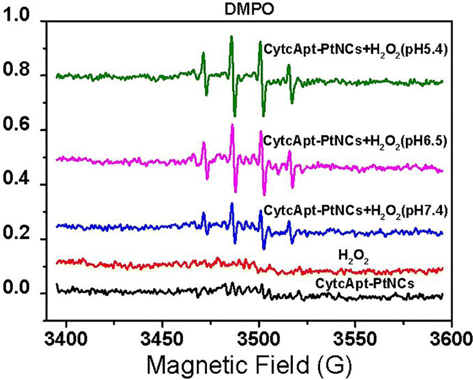
Fig. 5. ESR spectra of DMPO/⋅OH obtained from H2O2 with CytcApt-PtNCs at different pH values.
3.3. Cytotoxicity of CytcApt-PtNCs for normal cells
To test the cytotoxicity of CytcApt-PtNCs to normal cells, the viability of 293T cells treated with CytcApt-PtNCs was evaluated by standard MTT assay. As demonstrated in Fig. 6, no obvious cytotoxicity of CytcApt-PtNCs was observed, even the concentration was up to 40μM only. This result indicated that CytcApt-PtNCs have negligible cytotoxicity to 293T cells, suggesting the prominent biocompatibility of CytcApt-PtNCs.
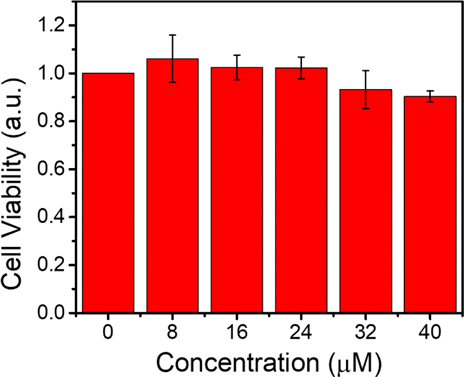
Fig. 6. Cell viability of 293T normal cells incubated with CytcApt-PtNCs.
3.4. Intracellular ROS detection of tumor cells
ROS generation ability catalyzed by CytcApt-PtNCs in 4T1 cells was studied using DCFH-DA as indicated. Figure 7 showed that strong green fluorescence was recorded when 4T1 tumor cells were incubated with CytcApt-PtNCs, which may be attributed to ROS production by catalyzing excessive H2O2 in tumor cells.
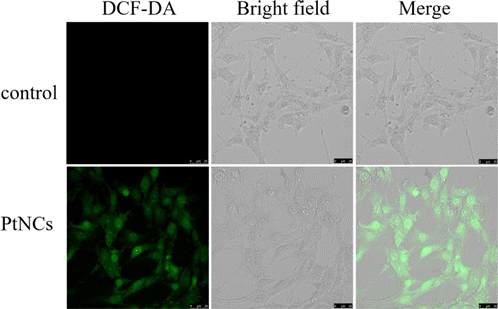
Fig. 7. Confocal fluorescence images of DCFH fluorescence in 4T1 cells. Scale bar: 50 μm.
3.5. Therapeutic effect of CytcApt-PtNCs on tumor cells
To test the effect of ⋅OH in cancer cells further, CDT performance of CytcApt-PtNCs was tested on tumor cells by MTT assay. The cytotoxicity of CytcApt-PtNCs was evaluated on 4T1 cells co-incubating with CytcApt-PtNCs at pH values of 5.4 (corresponding to the weak acidic microenvironment of lysosomes/endosomes), 6.5 (corresponding to the weak acidity in TME), and 7.4 (corresponding to physiological condition). Figure 8 showed that low-level CytcApt-PtNCs have negligible cytotoxicity to 4T1 cells at neutral condition (pH7.4). However, the survival rate of 4T1 cells reduced in a concentration-dependent manner under increasing acidic conditions. At the same concentration of CytcApt-PtNCs, the cytotoxic effect was higher in acidic environment (pH 6.5 and pH5.4) than under neutral conditions (pH7.4), indicating that CytcApt-PtNCs had a pH-dependent manner of CDT to 4T1 tumor cells. The results of MTT assays for 293T cells and 4T1 cells showed that CytcApt-PtNCs possessed a pH-dependent tendency to kill 4T1 tumor cells but had no side effect on normal 293T cells. Therefore, CytcApt-PtNCs possess excellent anti-cancer activity and selectivity in killing tumor cells.
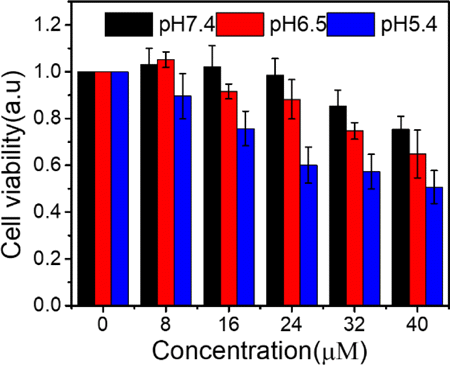
Fig. 8. Cell viability of 4T1 tumor cells incubated with CytcApt-PtNCs.
Live/Dead fluorescence cell co-staining assay was also performed to investigate the specific therapeutic effect of CytcApt-PtNCs in 4T1 cells at relatively low concentration. As shown in Fig. 9, CytcApt-PtNCs induced tumor cells death in pH-dependent tendency, which is consistent with the results of MTT assay. Therefore, these results together indicated that CytcApt-PtNCs possess excellent anti-tumor efficiency.
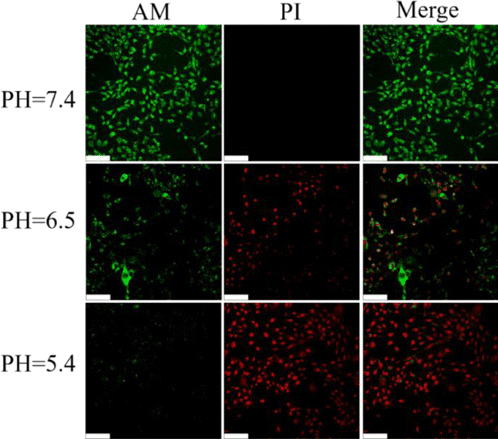
Fig. 9. Confocal fluorescence images of calcein AM (green, live cells) and PI (red, dead cells) contained 4T1 cells after coincubation with CytcApt-PtNCs at different pH. Scale bar:50 μm.
4. Conclusion
In this study, we first proposed a Fenton-like CDT agent based on CytcApt-PtNCs. The synthesized CytcApt-PtNCs can generate excessive ⋅OH in acid environment in 4T1 tumor cells. Moreover, CytcApt-PtNCs could kill 4T1 tumor cells in a pH-dependent tendency, but had no side effect on normal 293T cells. Therefore, CytcApt-PtNCs possess excellent anti-cancer activity and selectivity in killing tumor cells, which is promising for cancer treatment.
Conflicts of Interest
There are no conflicts to declare.
Acknowledgment
This work is supported by the Cross Research Fund of Biomedical Engineering of Shanghai Jiao Tong University (YG2019QNA43).