Development of water-soluble AIE-based wash-free Aβ probes superior to commercial ThT
Abstract
Alzheimer’s disease (AD) is a typical neurodegenerative disease. β-amyloid (Aβ) plaque is the most prominent pathological biomarker associated with the progression of AD. Conventional Aβ probes, including commercial probe ThT, usually suffer from tedious washing procedures. Herein, novel AIE-active Aβ probes with excellent water solubility, named DE-V1-PYC3 and DE-V1-PYOH, were developed for the detection and image of Aβ without tedious washing procedures. Compared with commercial probe ThT, the AIE-active Aβ probes exhibited better sensitivity and affinity to Aβ aggregates. Moreover, for ThT, the washing procedures are essential to obtain high signal-to-noise ratio (SNR) images of Aβ plaques in AD brain tissue slices. DE-V1-PYC3 and DE-V1-PYOH can label Aβ plaques in AD brain tissue slices with high SNR even without tedious washing procedures.
1. Introduction
Alzheimer’s disease (AD) is a typical neurodegenerative disease, which is characterized by memory impairment, aphasia, agnosia, and spatial cognition impairment.1,2,3 According to epidemiological research, it is estimated that by 2050, AD patients in the world will reach 152 million, and AD patients in China will reach 50 million, which will bring a huge economic burden to society and families.4 Although there are drugs for the treatment of AD, they only relieve the symptoms and cannot cure AD.3,5 So far, there have been no definitive opinions about the accurate pathogenesis and diagnosis of AD. It is well known that β-amyloid (Aβ) plaque is the most prominent pathological biomarker associated with the progression of AD.6,7,8 Therefore, the detection and image of Aβ plaques in the brain are essential to acquire more information about AD occurrence and development.
Fluorescence imaging is an efficient tool for Aβ detection due to its advantages of non-invasive, affordable, high contrast, etc.9,10,11,12 Therefore, many probes are designed to detect Aβ. Thioflavin T (ThT), a commercial probe, has been widely used for the diagnosis of Aβin vitro.13,14 In recent years, varied fluorescent Aβ probes have been developed for the detection of Aβin vitro and in vivo, such as thiosemicarbazone,15,16 curcumin,17,18,19,20 BODIPY,21,22,23 etc.24,25 However, such probes including ThT, usually have a strong binding capacity with biological tissues. This nonspecific binding to tissue makes strong background interference during imaging. Hence, a tedious rinsing process is needed to improve the signal-to-noise ratio (SNR). It is an urgent problem to develop wash-free Aβ probes.
Aggregation-induced emission (AIE) is a new fluorescence luminescence mechanism discovered by Tang in 2001.26 AIE-active probes emitted no emissions or weak fluorescence in the solution but exhibited strong fluorescence when combined with the target. Besides, AIE probes exhibited remarkable biocompatibility, resistance to photobleaching, high sensitivity and so on.27,28,29 Hence, AIE probes have been widely used in biosensing, bioimaging, etc.30,31,32 In recent years, amphiphilic/water-soluble AIE probes have emerged and been applied to wash-free imaging and in-situ tracing due to no fluorescence in the physiological environment.33,34 Therefore, amphiphilic/water-soluble AIE probe is a pathway to develop wash-free Aβ probes.
Based on ThT, new AIE-active water-soluble Aβ probes were designed. Aniline groups are employed as electron donor (D) and Aβ binding group, and pyridine cations acted as electron acceptor (A) and water-soluble groups. By bridging with carbon–carbon double bond (C=C), the water-soluble probes DE-V1-PYC3 and DE-V1-PYOH with D-A structure were constructed. They showed no emissions in physiological environment, but emitted strong fluorescence after binding to Aβ aggregates. The two AIE-active probes exhibited superior sensitivity and affinity to commercial probe ThT for Aβ, and labeled Aβ plaques in AD brain tissue slices with high SNR even without tedious washing procedures.
2. Materials and Methods
2.1. Materials and instruments
Unless specially stated, all solvents and chemicals were purchased from Bidepharm, Energy-chemical and Macklin. Aβ1−42 and Aβ1−40 peptides were purchased from Genicbio. All the animal experiments followed procedures approved by the Institutional Animal Ethics Committee of Huazhong University of Science and Technology.
1H NMR and 13C NMR Spectroscopy was performed on a Bruker AV 600 spectrometer or a Bruker AV 400 spectrometer at room temperature using DMSO-d6 or CDCl3 as the solvent. High-resolution mass spectrometry (HR-MS) was obtained with an Agilent 6550/Q-TOF instrument. The UV-Vis absorption and photoluminescence (PL) emission of the compounds were measured by UV-VIS Spectrophotometer (Shimadzu, UV-2600i) and fluorescence spectrometer (Edinburgh FLS1000), respectively. Fluorescence imaging was performed by a confocal laser-scanning microscope (Olympus FV3000, Japan).
2.2. Synthesis of DE-V1-PYC3 and DE-V1-PYOH
(E)-4-(4-(diethylamino) styryl)-1-propylpyridin-1-ium (DE-V1-PYC3) and (E)-4-(4-(diethylamino)styryl)-1-(2-hydroxyethyl)pyridin-1-ium (DE-V1-PYOH) were synthesized by Knoevenagel reaction. The detailed synthesis steps were as follows (Scheme 1).
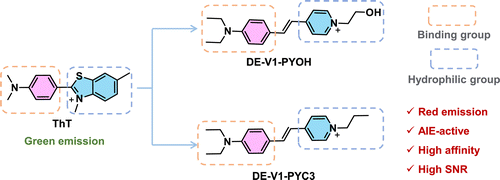
Scheme 1. Rational design of red emission AIE-active probes for Aβ aggregates based on ThT.
Synthesis of compound 1: A solution of 4-methylpyridine (5.1g, 54.83mmol) and 1-bromopropane (6.8g, 54.83mmol) in CH3CN (20 mL) was stirred at 80∘C for 16h. The solvent was evaporated under a vacuum to afford compound 1 (11.82g, crude), which is a brownish-yellow oil. It was used directly for the next step without further purification. 1H NMR (600MHz, DMSO-d6)δ8.94 (d, J=6.6Hz, 2H), 8.02 (d, J=6.6Hz, 2H), 5.17 (brs, 1H), 4.67 (t, J=4.8Hz, 2H), 3.85 (t, J=4.8Hz, 2H), 2.63 (s, 3H).
Synthesis of DE-V1-PYOH: Compound 1 and 4-(diethylamino) benzaldehyde were dissolved in ethanol (5mL). Then tetrahydropyrrole was added. The mixture was stirred at 80∘C for 16h. The solvent was evaporated under a vacuum. The residue was purified by recrystallization from EtOAc/EtOH to afford the target product, DE-V1-PYOH, which is a red solid. 1H NMR (400MHz, DMSO-d6)δ8.67 (d, J=7.1Hz, 2H), 8.04 (d, J=7.1Hz, 2H), 7.90 (d, J=16.0Hz, 1H), 7.57 (d, J=8.6Hz, 2H), 7.14 (d, J=16.0Hz, 1H), 6.75 (d, J=9.0Hz, 2H), 5.22 (t, J=5.2Hz, 1H), 4.46 (t, J=5.0Hz, 2H), 3.83 (q, J=5.1Hz, 2H), 3.43 (q, J=7.1Hz, 4H), 1.13 (t, J=7.0Hz, 6H). 13C NMR (101MHz, DMSO-d6)δ 154.37, 149.97, 144.34, 142.62, 131.09, 122.36, 122.23, 117.00, 111.87, 61.94, 60.53, 44.34, 12.97. HRMS (ESI, m/z): calcd for C19H25N2O (M–Br)+: 297.1961, found: 297.1968.
Synthesis of compound 2: A solution of 4-methylpyridine (5.0g, 54.83mmol) and 2-bioethanol (6.8g, 54.83mmol) in CH3CN (20mL) was stirred at 80∘C for 16h. The solvent was evaporated under a vacuum to afford compound 2 (11.82g, crude), which is a brownish-yellow oil. It was used directly for the next step without further purification. 1H NMR (400MHz, CDCl3)δ9.39 (d, J=6.7Hz, 2H), 7.92 (d, J=6.2Hz, 2H), 4.88 (t, J=7.3Hz, 2H), 2.68 (s, 3H), 2.09 (q, J=7.4Hz, 2H), 1.02 (t, J=7.4Hz, 3H).
Synthesis of DE-V1-PYC3: DE-V1-PYC3 was produced using the same procedure as DE-V1-PYOH by changing compound 1 to compound 2. DE-V1-PYC3 was obtained as bright red solid in 80% yield. 1H NMR (400 MHz, DMSO-d6)δ8.77 (d, J=6.9Hz, 2H), 8.06 (d, J=7.0Hz, 2H), 7.92 (d, J=16.1Hz, 1H), 7.58 (d, J=9.0Hz, 2H), 7.14 (d, J=16.0Hz, 1H), 6.76 (d, J=9.0Hz, 1H), 4.38 (t, J=7.2Hz, 2H), 3.43 (q, J=7.0Hz, 4H), 1.90 (q, J=7.3Hz, 2H), 1.13 (t, J=7.0Hz, 6H), 0.89 (t, J=7.4Hz, 3H). 13C NMR (101MHz, DMSO-d6)δ 154.30, 149.99, 143.89, 142.75, 131.10, 122.69, 122.24, 116.96, 111.88, 60.87, 44.34, 24.38, 12.97, 10.75. HRMS (ESI, m/z): calcd for C20H27N2 (M–Br)+: 295.2169, found: 295.2181.
2.3. Preparation of Aβ fibrils
Aβ1−42 (1mg) was dissolved in hexafluoroisopropanol (HPIF, 0.8mL), sonicated for 1min at room temperature to make it fully dissolved, and then vortexed for 1min to make it evenly dispersed. Then the HPIF-treated solution was incubated for 1h at room temperature. After cooling the solution on ice, the solvent was blown dry with nitrogen. 40μL of dimethyl sulfoxide (DMSO) and 1060μL of PBS buffer were added to the pretreated peptide membrane to form a 0.2mM monomer solution. Finally, the monomers were incubated for 4 days at 37∘C with constant speed stirring (300rpm) to obtain Aβ1−42 fibrils.35,36
2.4. In vitro colocalization imaging of Aβ plaques
The brain tissue slices of 5×FAD mouse (9 months, 100μm) were taken for in vitro fluorescent staining. After washing with PBS (10mM, pH=7.4) for 3 times, brain slices were incubated with 1% Thioflavin T (ThT) at room temperature for 5min. After washing with 50% ethanol/H2O for 3 times, the same slice was stained with DE-V1-PYC3 or DE-V1-PYOH (1μM, 10mM PBS) at room temperature for 5min and washed with PBS (10mM, pH=7.4) for 3 times. And then the slices were implemented for the fluorescence colocalization imaging test. Fluorescence colocalization imaging was performed by a confocal laser scanning microscope with the parameters as shown (for ThT, λex=405nm, λem=500–540nm; for DE-V1-PYC3 and DE-V1-PYOH, λex=488nm, λem=570–650nm). The images were analyzed by ImageJ software.
2.5. In vitro wash and wash-free imaging of Aβ plaques
The brain tissue slices of 5×FAD mouse (9 months, 100μm) were taken for in vitro wash and wash-free imaging of Aβ plaques. For ThT, the brain slices were washed with PBS (10mM, pH=7.4) for 3 times and then incubated with 1% ThT at room temperature for 5min. The staining brain sections were washed with 50% ethanol/H2O for 3 times and then PBS (10mM, pH=7.4) for another 3 times or wash-free before sealing. As for DE-V1-PYC3 and DE-V1-PYOH, the brain slices were washed with PBS (10mM, pH=7.4) for 3 times and then incubated with DE-V1-PYC3 or DE-V1-PYOH (1μM) at room temperature for 5min. The staining brain slices were washed with PBS (10mM, pH=7.4) for 3 times or wash-free before sealing. Fluorescence imaging was performed by a confocal laser scanning microscope with the parameters as shown (for ThT, λex=405nm, λem=500–540nm; for DE-V1-PYC3 and DE-V1-PYOH, λex=488nm, λem=570–650nm). The images were analyzed by ImageJ software.
3. Result and Discussion
3.1. Synthesis and photophysical properties of DE-V1-PYC3 and DE-V1-PYOH
The target compounds DE-V1-PYC3 and DE-V1-PYOH were characterized by 1H NMR, 13C NMR, and HRMS (Figs. S1–S8). Considering that the fluorescent labeling was performed in the aqueous medium, DE-V1-PYC3 and DE-V1-PYOH were designed by introducing a pyridine cation group with the expectation of enhancing their hydrophilicity. The lipid–water partition coefficient (logP) is an important index to characterize the hydrophilicity and hydrophobicity of compounds. The lipid-water partition coefficient (logP) of DE-V1-PYC3 and DE-V1-PYOH in n-octanol/water was measured as 0.066 and −0.21, respectively, demonstrating that they are water-soluble compounds (Table S1). The UV–Vis absorption and photoluminescence (PL) spectra of ThT, DE-V1-PYC3 and DE-V1-PYOH were measured in an aqueous solution. The maximum absorption peak and emission peak of ThT were located at ∼410nm and ∼480nm, respectively. Compared with ThT, the maximum absorption peaks of DE-V1-PYC3 and DE-V1-PYOH were located at ∼480nm, which red-shifted ∼70nm due to the extension of the conjugated structure. While their emission peaks were located at ∼630nm, which red-shifted ∼150nm compared with ThT (Fig. 1). Compared with the 70nm Stokes shifts of ThT, DE-V1-PYC3 and DE-V1-PYOH exhibited large Stokes shifts as 150nm, which is beneficial to reduce self-absorption and improve luminescence efficiency.
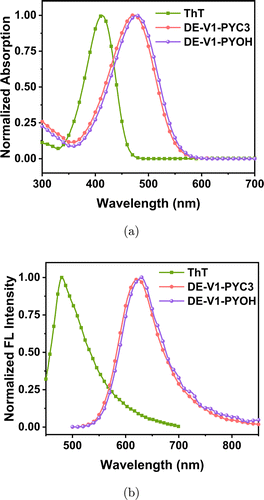
Fig. 1. (a) UV–Vis absorption spectra of ThT, DE-V1-PYC3 and DE-V1-PYOH in water. (b) Emission spectra of ThT, DE-V1-PYC3 and DE-V1-PYOH in water. Dye concentration: 10μM.
3.2. Aggregation-induced emission characteristic study
Since the probes DE-V1-PYC3 and DE-V1-PYOH are water-soluble compounds, they are soluble in polar solvents water and poorly soluble in low polar solvents tetrahydrofuran (THF). Hence, the AIE characteristics were investigated in THF/water mixtures with different tetrahydrofuran (THF) fractions (Fig. 2). The probes showed feeble emission in water due to the non-radiative transition. When the poor solvent THF was added, the fluorescence turned on due to radiative transition activated by restriction of intramolecular motions (RIM). The fluorescence was gradually enhanced with the increase of THF content. When the THF content was increased to 99%, the fluorescence of DE-V1-PYC3 and DE-V1-PYOH was significantly enhanced with the emission enhanced about 25-fold and 79-fold compared in water, respectively. The results demonstrated that both DE-V1-PYOH and DE-V1-PYC3 are typical AIE-active water-soluble molecules. The negligible fluorescence of the probes in water is conducive to the “off–on” detection in biological detection with weak background interference.
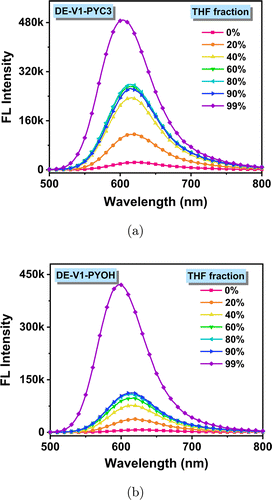
Fig. 2. Emission spectra of DE-V1-PYC3 (a) and DE-V1-PYOH (b) in THF/water mixtures with different THF fractions. Dye concentration: 10μM.
3.3. Sensitivity and affinity of water-soluble AIEgens to Aβ fibrils
The response of the probes to Aβ fibrils was tested with Aβ1−42 fibrils in PBS (10mM, pH=7.4). As water-soluble compounds, DE-V1-PYC3 and DE-V1-PYOH emitted negligible fluorescence at ∼630nm in PBS (10mM, pH=7.4). While a trace amount of Aβ fibrils was added, the fluorescence turned on with red emission at ∼605nm. The 25nm blue-shift of the emission peak may be due to the probes entering the hydrophobic cavity of Aβ fibrils. After the addition of 10μM Aβ1−42 fibrils, the fluorescence intensity of ThT increased nearly 16-fold (Fig. S9). Compared with ThT, the fluorescence intensity of DE-V1-PYC3 and DE-V1-PYOH increased nearly 22-fold and 18-fold, respectively (Figs. 3(a) and 3(b)). The results showed that both DE-V1-PYC3 and DE-V1-PYOH possessed excellent sensitivity to Aβ1−42 fibrils. Moreover, the selectivity of DE-V1-PYC3 and DE-V1-PYOH for Aβ fibers was also evaluated with amino acids, metabolic substances, and associated monomers as the potentially competitive species. The results showed that DE-V1-PYC3 and DE-V1-PYOH show minimal fluorescence response to the potentially competitive species, but strong response to Aβ fibers, indicating excellent selectivity to Aβ fibrils (Fig. S10).
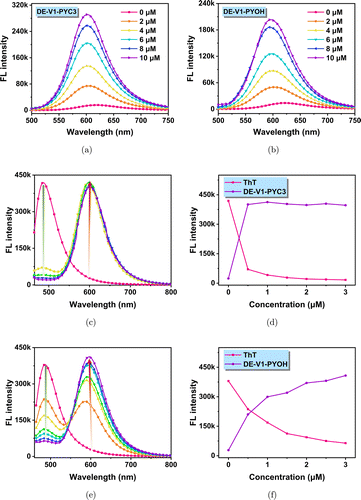
Fig. 3. Emission spectra of DE-V1-PYC3 (a) and DE-V1-PYOH (b) in PBS solution with different Aβ1−42 fibrils content (dye concentration: 2μM). DE-V1-PYC3 (c) and DE-V1-PYOH (e) effectively replace ThT from the ThT/Aβ1−42 fibrils complex in PBS. The emission intensity of the peaks at 486nm and 605nm with the concentration of DE-V1-PYC3 (d) and DE-V1-PYOH (f).
To confirm the affinity of water-soluble AIEgens to Aβ fibrils, the competitive experiment was carried out to compare the affinity of the probe DE-V1-PYC3 and DE-V1-PYOH with the commercial Aβ probe ThT. ThT/Aβ1−42 aggregates were prepared by pre-mixed ThT (5μM) with Aβ aggregates (10μM), which showed strong green emission at ∼486nm. When DE-V1-PYC3 (0–3μM) was gradually added, the fluorescence of ThT at 486nm sharply weaken, while the fluorescence of DE-V1-PYC3 at 605nm dramatically increased (Figs. 3(c) and 3(d)). When DE-V1-PYOH (0–3μM) was gradually added, the fluorescence of ThT at 486nm gradually weakens, while the fluorescence of DE-V1-PYOH at 605nm gradually increased (Figs. 3(e) and 3(f)). This is probably due to DE-V1-PYC3/DE-V1-PYOH replacing ThT from ThT/Aβ1−42 aggregates, indicating that DE-V1-PYC3 and DE-V1-PYOH own better affinity with Aβ aggregates than commercial probe ThT.
Furthermore, we investigated the binding model of ThT, DE-V1-PYC3 and DE-V1-PYOH to Aβ by simulation calculation using Schrodinger software. The results showed that all three molecules could be inserted into the β-sheet cavity of Aβ, which restricted the motion of the molecules (Fig. 4). Hence, ThT, DE-V1-PYC3 and DE-V1-PYOH showed excellent fluorescence response to Aβ due to restriction of intramolecular motions (RIM). Besides, DE-V1-PYC3 and DE-V1-PYOH also interact with the amino acid residues of Aβ. DE-V1-PYC3 showed the C–H⋯O interaction with the residue of asparagine (Asn) and aspartic acid (Asp), and the N+⋯O interaction with the residue of aspartic acid (Asp) (Fig. 4(b)). While DE-V1-PYOH showed the C–H⋯O, O–H⋯O, N+⋯O interactions with the residue of aspartic acid (Asp) (Fig. 4(c)). These interactions indicated that DE-V1-PYC3 and DE-V1-PYOH own better affinity with Aβ than commercial probe ThT.
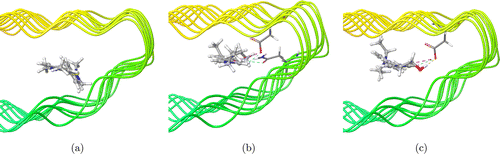
Fig. 4. Three-dimensional models of ThT (a), DE-V1-PYC3 (b), and DE-V1-PYOH (c) were binding to Aβ fibrils. The dashed lines represent the interaction of the molecule with amino acid residues of Aβ fibrils, the yellow dashed lines represent hydrogen bonding interactions, and the pink dashed lines represent salt bridges.
3.4. In vitro colocalization imaging of Aβ plaques with commercial probe ThT
To verify the target property of DE-V1-PYC3 and DE-V1-PYOH for Aβ plaques, the two AIE probes were applied for staining Aβ plaques in brain tissue of AD mouse (5×FAD, 9 months). The brain sections were stained with DE-V1-PYC3 and DE-V1-PYOH (red channel), and then co-incubated with commercial probe ThT (green channel). The colocalization imaging showed that the red fluorescent spots emitted by DE-V1-PYC3 almost overlapped with the green fluorescent spots of ThT with the Pearson’s correlation coefficient (Rp) as 0.91 (Figs. 5(a)–5(d)). Similarly, the red fluorescent spots emitted by DE-V1-PYOH almost overlapped with the green fluorescent spots of ThT with the Pearson’s correlation coefficient (Rp) as 0.96 (Figs. 5(e)–5(h)). Furthermore, DE-V1-PYC3 and DE-V1-PYOH were employed to stain the brain sections from wild-type mouse (9 months). No significant signal was observed, demonstrating that DE-V1-PYC3 and DE-V1-PYOH are excellent and special probes for Aβ plaques (Fig. S11).
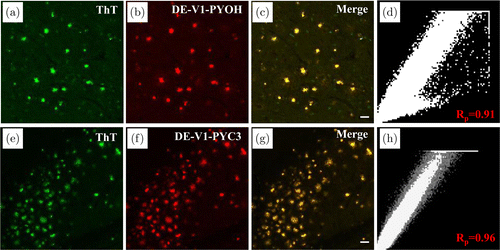
Fig. 5. Fluorescence imaging of Aβ plaques in brain tissue sections from AD mouse. (a) (e) ThT staining, (b) DE-V1-PYOH staining, and (f) DE-V1-PYC3 staining. (c) (g) The merged images. (d) (h) The Pearson correlation coefficient analysis. Scale bar: 50μm.
3.5. In vitro wash and wash-free imaging of Aβ plaques
Most reported Aβ probes need to wash away excess nonspecific binding dyes to the tissue with solvent or PBS after labeling to obtain images with a high signal-to-noise ratio (SNR). DE-V1-PYOH and DE-V1-PYC3 are excellent water-soluble AIE-active probes with remarkable sensitivity and affinity for Aβ. Due to intrinsic AIE properties, DE-V1-PYOH and DE-V1-PYC3 show no fluorescence in physiological media, and only emit strong fluorescence after binding to Aβ. Besides, due to good water solubility, nonspecific binding of the probes to tissue is reduced. Hence, tedious washing procedures may be redundant after staining with DE-V1-PYOH and DE-V1-PYC3. The wash and wash-free tests of AD brain tissue sections staining with ThT, DE-V1-PYOH and DE-V1-PYC3 were carried out. For ThT, the wash-free tissue section showed strong background interference with a low SNR as ∼4. After washing with 50% EtOH/H2O, the background fluorescence significantly decreased with a high SNR as ∼15. For DE-V1-PYOH and DE-V1-PYC3, both the wash-free and wash tissue sections showed negligible background fluorescence with strong signals. The SNR was calculated as ∼14 and ∼18 for DE-V1-PYOH and DE-V1-PYC3, respectively. The results demonstrated that compared with ThT, DE-V1-PYOH and DE-V1-PYC3 could label Aβ plaques without tedious washing procedures.
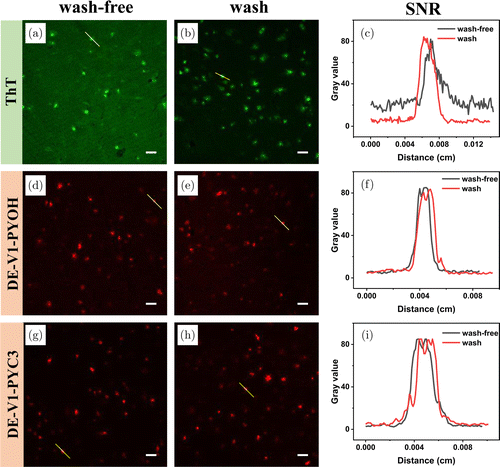
Fig. 6. Washing-free and wash imaging studies of ThT (a–c), DM-V1-PYOH (d–f) and DM-V1CN-PYOH (g–i). (a) (d) (g) washing-free, (b) (e) (h) washing. (c) (f) (i) the SNR of the yellow line areas. Scale bar: 50μm.
4. Conclusion
In conclusion, we successfully designed and constructed two AIE-active probes named DE-V1-PYC3 and DE-V1-PYOH with excellent water solubility for the detection of Aβ. The significant AIE probes exhibited remarkable turn-on fluorescence enhancements, great sensitivity and affinity to Aβ aggregates. In addition, DE-V1-PYC3 and DE-V1-PYOH own stronger affinity for Aβ fibrils than ThT. They demonstrate remarkable targeting ability for Aβ plaques in AD brain tissue sections. Moreover, the two AIE-active Aβ probes can label Aβ plaques with high SNR even without tedious washing procedures. This work provides a new idea for the design of novel probes for the detection of Aβ.
Acknowledgments
We thank Xiangning Li and Hui Gong from the Huazhong University of Science and Technology for providing the brain tissue of 5xFAD mouse. This work was supported by the National Key R&D Program of China (2021ZD0201004), the National Natural Science Foundation of China (22165008, 22077037, 21474034, 51673077 and 51603078), Hainan Provincial Natural Science Foundation of China (521RC506), the Open Project Program of Wuhan National Laboratory for Optoelectronics (No. 2020WNLOKF018). We also thank the Analytical and Testing Center of the Hainan University for use of their facilities.
Supplementary Information
The Supplementary Information are available at: https://www.worldscientific.com/doi/suppl/10.1142/S1793545823300094.
Conflict of Interest
The authors have no conflicts of interest relevant to this article.