Brain-wide N2cG compensation permits glycoprotein-deleted rabies virus to trace neural circuits across multiple synapses
Abstract
Rabies-viruses-based retrograde tracers can spread across multiple synapses in a retrograde direction in the nervous system of rodents and primates, making them powerful tools for determining the structure and function of the complicated neural circuits of the brain. However, they have some limitations, such as posing high risks to human health and the inability to retrograde trans-synaptic label inputs from genetically-defined starter neurons. Here, we established a new retrograde trans-multi-synaptic tracing method through brain-wide rabies virus glycoprotein (RVG) compensation, followed by glycoprotein-deleted rabies virus (RV-G) infection in specific brain regions. Furthermore, in combination with the avian tumor virus receptor A (TVA) controlled by a cell-type-specific promoter, we found that EnvA-pseudotyped RV-G can mediate efficient retrograde trans-multi-synaptic transduction from cell-type-specific starter neurons. This study provides new alternative methods for neuroscience researchers to analyze the input neural networks of rodents and nonhuman primates.
1. Introduction
Analyzing the input and output pathways of the complex neural circuits in the brain is the basis of revealing the principles of the brain function and elucidating the mechanisms through which brain diseases occur.1,2 Retrograde tracers based on neurotropic viruses have been continuously developed to achieve structural imaging and functional manipulation of input networks in different model animals, providing valuable gene delivery tools for research in neuroscience and gene therapy.3,4,5,6,7
Retrograde viral tracers include retrograde trans-synaptic viruses and retrograde non-trans-synaptic viruses. The commonly used retrograde viral tracers are adeno-associated virus (AAV),6,8 lentivirus (LV),9 canine adenovirus (CAV),10 rabies virus (RV),11 herpes simplex virus (HSV),12 and pseudorabies virus (PRV).13 Among these viruses, only the first three viruses cannot be retrogradely transmitted across synapses. The toxicity of retrograde non-trans-synaptic viruses is generally lower than that of trans-synaptic virus, and some of them are used as gene therapeutic vectors. However, the retrograde non-trans-synaptic viruses cannot be directly used to trace the whole-brain synaptic inputs from cell-type-specific starter cells, whereas the retrograde trans-synaptic viruses can. Retrograde non-trans-synaptic viruses are mainly used to study the structural and functional networks of projection neurons; moreover, they can be used together with retrograde trans-synaptic viruses, enabling trans-synaptic input tracing from specific subsets of neurons based on their projection and cell type.8,14
RV and PRV Bartha are retrograde trans-multi-synaptic viral vectors that can be used to map multiple input networks in the peripheral and central nervous systems.15 One important feature of RV is that it can infect primates, whereas PRV Bartha cannot, so RV is a powerful viral tool for dissecting the complex neural connections within the nervous system, especially in nonhuman primates.16,17 In general, wild-type RV is deadly to both humans and other animals; thereby, extreme caution is required for its use: Researchers working with it need to be vaccinated.16 Because PRV Bartha does not infect humans, recombinant PRV Bartha has been preferentially used for the neural circuit analysis of the peripheral and central nervous systems in rodents.18 For the precise identification of special neural circuits, PRV-Ba2001, a Cre-dependent tracer that can be retrogradely transmitted from originally infected cell-type-specific neurons, was developed.19 However, strategies based on Cre-dependent DNA recombination for cell-type-specific labeling cannot be applied to RV, because RV is an RNA virus and has no DNA phase in the viral life cycle.16 In addition, RV and PRV exhibit differential infection tropism in the central nervous system of rodents.20 Therefore, RV-based retrograde multi-trans-synaptic technology needs to be developed.
Several attenuated rabies viral vectors have been developed, such as the SAD-B1921,22 and CVS-N2c23 strains. Glycoprotein-deleted rabies virus (RV-G) is deficient in replication and thus cannot propagate beyond the originally infected neurons, unless RV glycoprotein (RVG) is supplied.11 Based on this, a RV-G-based retrograde trans-monosynaptic system was developed.21 CVS-N2c-G exhibits a reduction in neuronal toxicity and a substantial enhancement in trans-synaptic neuronal transfer compared with the SAD-B19-G vaccine strain.23,24 RV-G pseudotyped with avian sarcoma and leukosis virus A protein (EnvA) can only infect cells that express the avian tumor virus receptor A (TVA); according to this, specific infection can be achieved.15,21
In this study, through brain-wide N2cG compensation using AAV-PHP.eB, followed by CVS-N2c-G infection in specific brain regions, we established a new retrograde trans-multi-synaptic tracing method. Furthermore, in combination with the avian TVA controlled by a cell-type-specific promoter, we achieved retrograde trans-multi-synaptic tracing from cell-type-specific starter neurons. Our study provides new alternative methods for neuroscience researchers to analyze the input neural networks of rodents and nonhuman primates.
2. Materials and Methods
2.1. Plasmid construction and viral vector preparation
To construct pAAV-EF1-N2cG-WPRE-pA, we amplified N2cG gene sequence by PCR, which we inserted into DIO-EF1a-FLEX-H2B-GFP-P2A-N2cG (Addgene plasmid #73476) digested by restriction enzymes SalI and EcoRI (New England Biolabs). pAAV-CaMKII-EGFP-T2A-TVA-WPRE-pA was provided as a gift from BrainCase Biotechnology Co., Ltd. (ShenZhen, China). We produced the AAV vectors, including rAAV-PHP.eB-EF1-N2cG-WPRE-pA and rAAV9-CaMKII-EGFP-T2A-TVA-WPRE-pA, by triple-plasmid transient transfection in HEK293T cells, pUCmini-iCAP-PHP.eB (Addgene plasmid #103005) or pAAV2/9n (Addgene plasmid #112865) as the packaging plasmid and pAdDeltaF6 (Addgene plasmid #112867) as the adenovirus element helper plasmid. The virus purification and concentration procedures were performed as previously described.3,5,25 The titers of rAAV-PHP.eB-EF1-N2cG-WPRE-pA and rAAV9-CaMKII-EGFP-T2A-TVA-WPRE-pA were viral genomes/mL (VG/mL) and VG/mL, respectively. We prepared B19G-CVS-N2c-G-EGFP and EnvA-CVS-N2c-G-tdTomato according to a previously reported method.24 The titers of B19G-CVS-N2c-G-EGFP and EnvA-CVS-N2c-G-tdTomato were infectious units per mL(IU/mL) and IU/mL, respectively.
2.2. Research animals
All surgical and experimental procedures were approved by the Animal Care and Use Committee of Innovation Academy for Precision Measurement Science and Technology, Chinese Academy of Sciences. We used adult C57BL/6 mice (Hunan SJA Laboratory Animal Company, Changsha, China) for all experiments. We housed the mice in the appropriate environment with a 12/12h light/dark cycle; we supplied water and food ad libitum.
2.3. Viral vector injection
We selected the stereotactic injection coordinates according to Paxinos and Franklin’s The Mouse Brain in Stereotaxic Coordinates, 4th edition.26 The stereotactic coordinates for the MOp were as follows: Anterior–posterior (AP) axis: mm; medial–lateral (ML) axis: mm; and dorsal–ventral (DV) axis: mm from the bregma; we performed the stereotactic injection process as previously reported.5 For the intravenous administration, we injected the C57BL/6 mice with rAAV-PHP.eB-EF1-N2cG-WPRE-polyA at a dose of VG/mouse.
2.4. Slice preparation and imaging
We prepared and imaged the slices according to previously reported methods.8 We soaked the brains overnight in 4% paraformaldehyde solution. After we completed dehydration with 30% sucrose solution, we sectioned the brains into m slices with a microtome (Thermo Fisher Scientific, Waltham, MA, USA), which we collected in antifreeze fluid and stored at C until further use. After thorough washing with PBS, we attached all the brain slices to microscope slides, which we then counterstained with DAPI (1:4000) and sealed with 70% glycerol. For imaging, we used a virtual microscopy slide-scanning system (Olympus, VS 120, Tokyo, Japan).
3. Results and Discussion
3.1. Establishment of novel retrograde trans-multi-synaptic tracing methods
We previously reported that brain-wide TVA receptor compensation allows rabies virus to retrograde target projection neurons of a specific brain region.5 Based on similar principles, we used AAV-PHP.eB to achieve brain-wide compensation of RVG, and designed a RV-G-based retrograde trans-multi-synaptic system. The functional elements carried in the AAVs and RV-G are illustrated in Figs. 1(a) and 1(b), respectively. Based on our previous findings,24 we selected the CVS-N2c strain of rabies virus for this study. For retrograde trans-multi-synaptic labeling, we used an AAV virus for brain-wide N2cG compensation and a B19G-pseudotyped CVS-N2c-G (B19G-CVS-N2c-G) (Fig. 1(c)). For retrograde trans-multi-synaptic labeling from cell-type-specific starter cells, we used an AAV virus for brain-wide N2cG compensation, an AAV virus carrying TVA controlled by a cell-type-specific promoter, and an EnvA-pseudotyped CVS-N2c-G (EnvA-CVS-N2c-G) (Fig. 1(d)).
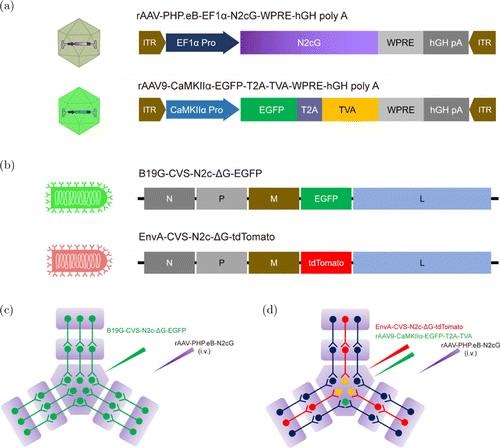
Fig. 1. Schematic diagram of viral vectors and design of retrograde trans-multi-synaptic tracing. (a) AAVs used for retrograde trans-multi-synaptic tracing: rAAV-PHP.eB for carrying N2cG controlled by EF1 promoter, and rAAV9 for carrying TVA controlled by CaMKII promoter. (b) RVs used for retrograde trans-multi-synaptic tracing: B19G-CVS-N2c-G-EGFP for retrograde trans-multi-synaptic tracing, and EnvA-CVS-N2c-G-tdTomato for retrograde trans-multi-synaptic tracing from genetically defined starter neurons. EnvA-CVS-N2c-G-tdTomato could specifically recognize and infect TVA-positive neurons. (c) Schematic diagram of retrograde trans-multi-synaptic tracing strategy. i.v., intravenous injection. (d) Schematic diagram of genetically defined retrograde trans-multi-synaptic tracing strategy. i.v., intravenous injection.
3.2. New method enables efficient trans-monosynaptic labeling
To verify whether the helper virus could effectively mediate the trans-synaptic tracing of RV-G, we first used the rAAV-PHP.eB vector for in situ delivery of the N2cG gene. We injected rAAV-PHP.eB-EF1-N2cG into the primary motor area (MOp); after three weeks, we injected B19G-CVS-N2c-G-EGFP at the same site (Fig. 2(a)). Five days later, we sacrificed the mice, and we imaged the upstream inputs of the MOp. We observed many EGFP-positive neurons in the brain regions, such as in the ventral anterior–lateral complex of the thalamus (VAL), the superior colliculus, motor-related (SCm), the substantia nigra, reticular (SNr), and the dentate nucleus (DN) (Fig. 2(b)). These results verified the effect of AAV-PHP.eB as a helper virus.
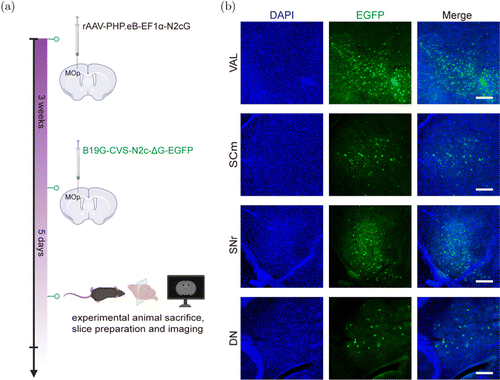
Fig. 2. Local injection to verify trans-synaptic effect. (a) rAAV-PHP.eB-EF1-N2cG was injected in primary motor area (MOp) of C57BL/6 mice; at three weeks postinjection, B19G-CVS-N2c-G-EGFP was injected into the same site, and mice were sacrificed after five days. (b) Fluorescence expression in brain regions upstream of MOp. VAL, ventral anterior–lateral complex of the thalamus; SCm, superior colliculus, motor-related; SNr, substantia nigra, reticular; DN, dentate nucleus. Scale bar m.
3.3. CVS-N2c-G-based retrograde trans-multi-synaptic tracing
To trace the upstream input networks of a special brain region, N2cG was carried by rAAV-PHP.eB and compensated in the whole brain via intravenous administration. Three weeks after expression, we injected B19G-CVS-N2c-G-EGFP in the MOp (Fig. 3(a)). Five days later, we sacrificed the mice, and we imaged the upstream inputs of the MOp. We found that B19G-CVS-N2c-G-EGFP infected the injection site (Fig. 3(b)) and retrograde trans-synaptic label the upstream brain regions (Figs. 3(b) and 3(c)), including the VAL, basolateral amygdalar nucleus (BLA), subiculum (SUB), SNr, simple lobule (SIM), DN, and caudate putamen (CPu), among others. The CPu was proved to be an indirect input of the MOp.27,28 These results indicated that CVS-N2c-G-based retrograde trans-multi-synaptic tracing technology can be used to label brain-wide input networks of specific brain regions. When tracing neural circuits in the motor systems of nonhuman primates, the CVS-N2c strain of rabies virus can rapidly spread across one synaptic step every 10–12h.16,29,30 Thus, CVS-N2c-G also has the potential to attain time-dependent retrograde trans-synaptic labeling. In addition, using CVS-N2c-G vector to express a slow fluorescent timer (sFT), whose fluorescence color can change from blue to red over time,2,31 will allow us to distinguish first-, second-, and third-order neurons according to the red-to-blue fluorescence intensity ratio.
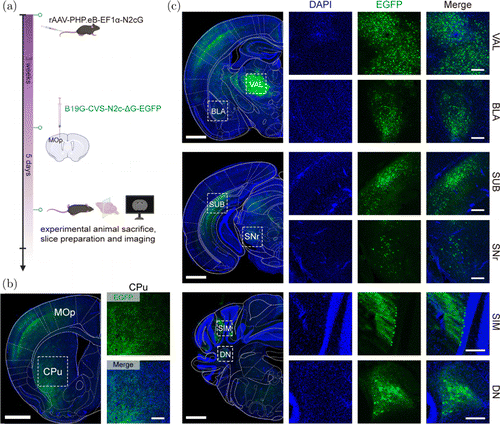
Fig. 3. CVS-N2c-G-based retrograde trans-multi-synaptic tracing at five days postinfection. (a) Schematic diagram of retrograde trans-multi-synaptic tracing. Helper virus expressing N2cG was intravenously administered; after three weeks, the B19G-CVS-N2c-G-EGFP was injected into the primary motor area (MOp). Then mice were sacrificed after five days. (b) Fluorescence expression at injection site (MOp), and upstream brain region caudate putamen (CPu). In large picture on left, scale bar 1mm; in small pictures on right, scale bar m. (c) Fluorescence expression in upstream brain regions. VAL, ventral anterior–lateral complex of the thalamus; BLA, basolateral amygdalar nucleus; SUB, subiculum; SNr, substantia nigra; SIM, reticular simple lobule; DN, dentate nucleus. Large picture on left, scale bar mm; small pictures on right, scale bar m.
3.4. CVS-N2c-G-based retrograde trans-multi-synaptic tracing from genetically defined starter neurons
To further trace the input networks of excitatory neurons in the MOp, we administered rAAV9 carrying TVA and EGFP under the control of the CaMKII promoter into the MOp. Concomitantly, we used rAAV-PHP.eB for brain-wide N2cG compensation via tail vein injection. After three weeks, we injected EnvA-CVS-G-tdTomato into the MOp (Fig. 4(a)). Five days later, we sacrificed the mice, and we imaged the upstream inputs of the MOp. We observed TVA-positive cells (rAAV, green signals) and tdTomato-positive cells (RV, red signals) in the MOp (Fig. 4(b)). Many upstream brain regions were also labeled by CVS-G-tdTomato (Figs. 4(b) and 4(c)), such as the anterior cingulate area (ACA), VAL, SCm, SNr, and CPu, among others. These results demonstrated that using EnvA-pseudotyped RV-G in combination with rAAV-PHP.eB carrying TVA controlled by a cell-type-specific promoter, retrograde trans-multi-synaptic tracing from genetically defined starter neurons can be achieved. Based on this principle, researchers can also use a Cre-dependent AAV vector expressing TVA, combined with Cre transgenic animals, to create a strategy for the retrograde trans-multi-synaptic tracing from genetically defined starter neurons. Notably, using our strategies to trace the neural circuits of nonhuman primates, AAVs that are more efficient across the blood–brain barrier in nonhuman primates are required. The AAVs may be AAV-B10,32 AAV.CPP.16,33 etc.
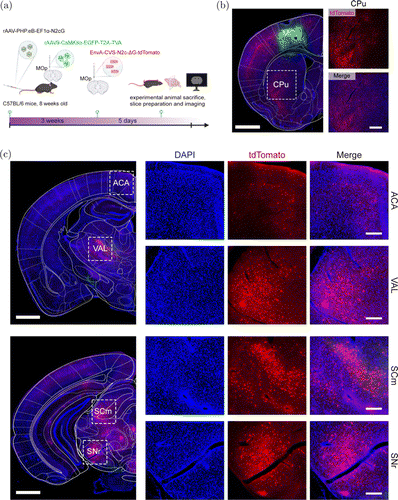
Fig. 4. CVS-N2c-G-based starting-cells-specific retrograde trans-multi-synaptic tracing. (a) Schematic diagram of starting-cells-specific retrograde trans-multi-synaptic tracing. rAAV-PHP.eB expressing N2cG was intravenously administered, and rAAV9 expressing TVA was injected in primary motor area (MOp); after three weeks, EnvA-CVS-N2c-G-tdTomato was injected at same site, then mice were sacrificed after five days. (b) Green fluorescence expression at injection site (MOp), and tdTomato fluorescence expression at the upstream brain region caudate putamen (CPu). Large picture on left, scale bar 1mm; small pictures on right, scale bar m. (c) tdTomato fluorescence expression in upstream brain regions. ACA, anterior cingulate area; VAL, ventral anterior–lateral complex of the thalamus; SCm, superior colliculus, motor-related; SNr, substantia nigra. Large picture on left, scale bar mm; small pictures on right, scale bar m.
4. Conclusions
Using a BBB-crossing rAAV-PHP.eB as a helper virus for brain-wide compensation of RVG, RV-G can be used to retrograde trace neural circuits of specific brain regions across multiple synapses. Furthermore, in combination with TVA controlled by a cell-type-specific promoter, RV-G pseudotyped with EnvA enables effective retrograde trans-multi-synaptic spreading from cell-type-specific starter neurons. This study provides new approaches for identifying the input neural networks of rodents and nonhuman primates.
Acknowledgments
This study was supported by the STI2030-Major Projects (Grant No. 2021ZD0201003), the National Natural Science Foundation of China (Grant Nos. 31830035, 31771156, 21921004, and 32100899), the Strategic Priority Research Program of the Chinese Academy of Sciences (Grant No. XDB32030200), and the Shenzhen Key Laboratory of Viral Vectors for Biomedicine (Grant No. ZDSYS20200811142401005), the Key Laboratory of Quality Control Technology for Virus- Based Therapeutics, Guangdong Provincial Medical Products Administration (Grant No. 2022ZDZ13), and Open Project Program of Wuhan National Laboratory for Optoelectronics (Grant No. 2019WNLOKF022).
We are grateful to Liting Luo and Lingling Xu (Core Facility Center, Innovation Academy for Precision Measurement Science and Technology, Chinese Academy of Sciences) for technical assistance with centrifugation and microscope imaging. The schematic diagrams in this paper were created with BioRender.com.
Conflicts of Interest
The authors declare that there are no conflicts of interest relevant to this paper.